The UK is rapidly shifting from a fossil-fuel based economy towards cleaner energy sources. Technologies central to this transition-including wind turbines (WT), photovoltaics (PV) and electrical vehicles (EV) - in many cases make use of a group of 17 elements collectively referred to as the 'rare earth elements' (REE).
Future of electric vehicles (EV)
UK sales of electric vehicles (EVs) are growing rapidly supported by a range of policy incentives including purchase grants, licensing and charge breaks and tax benefits. Their market share is anticipated to grow further in line with a UK government commitment to end sales of new petrol and diesel vehicles by 2030.
To achieve decarbonisation in the transport sector, new value chains will need to be established and reconfigured, with implications for importing, processing and revalorisation of critical raw materials. In this section, we present information on the current and future state of embedded materials and potential demand/supply challenges for EVs.
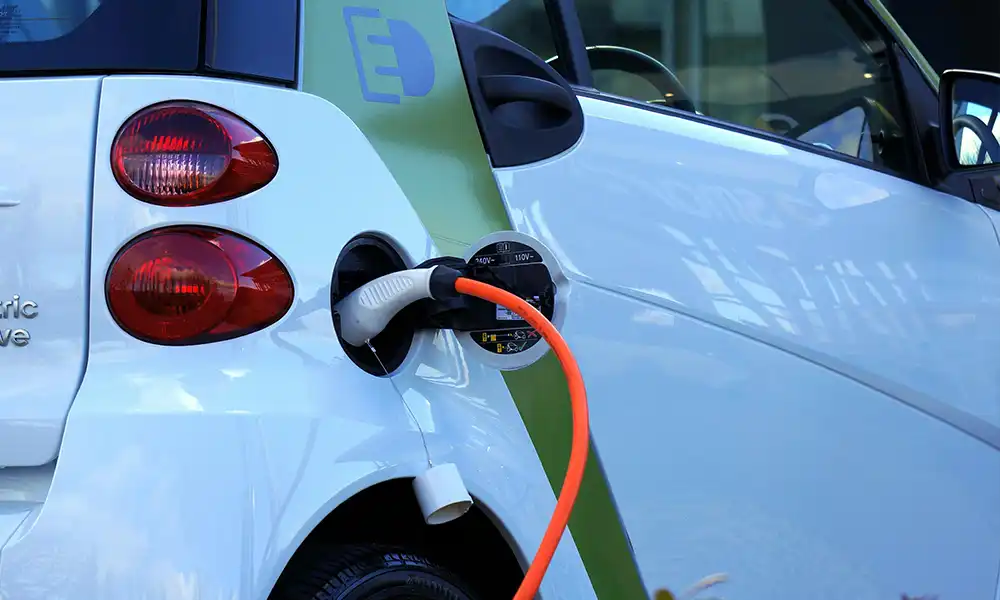
Components and metals in electrical vehicles
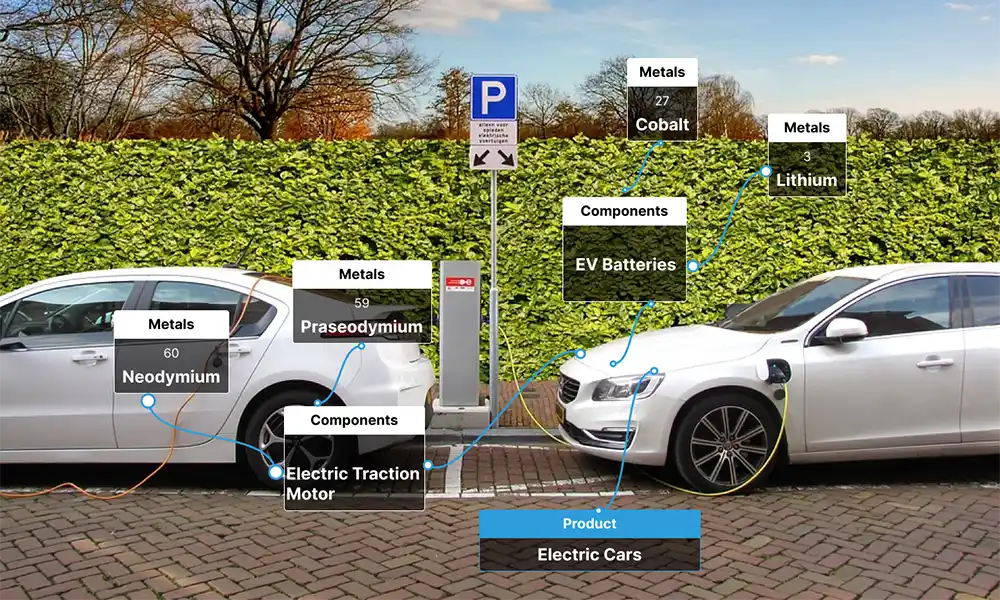
Demand
The number of licensed ultra-low emissions vehicles in the UK stood at just short of 1 million vehicles in 2022, around 8 times higher than in 2017. While representing only around 2% of all vehicles registered in the UK, their share of the market of new vehicles registered was far greater at roughly a fifth (DfT).
This share is expected to grow and with it, material demand such as for rare earth elements - the structural, physical and chemical properties of which have cemented their place as increasingly technology-critical across a range of applications. These include in magnets, catalysts and batteries (Cobelo-García et al. 2015; Zhou, 2017; Dang et al. 2021).
The UK is not alone in its aims for a low-carbon energy transition. The IEA (2020) estimates that to meet stated policy ambitions, global demand for REEs will increase by almost four times between 2020 and 2040.
Supply
The prefix 'rare' is somewhat of a misnomer for REEs given their relatively high abundance in the lithosphere. Low concentrations render extraction commercially unviable in many areas however, and the UK is effectively 100% import dependent for these elements.
REE supply to the global market is concentrated in only a few countries. China, who whilst representing approximately 54% of global mining supply in 2021 and were the source of approximately 95% of refined supply (Roskill in Liang, 2021). 96%of the global REE reserves (120 million metric tons) is concentrated in six countries, including China (38%),Vietnam (19%), Brazil (18%), Russia (10%), India (6%),and Australia (5%) (Gambogi 2021).
Complex supply chain involving the production of specialist alloys, then magnet powder, magnets themselves and assembly into EVs.
Prices and costs
With UK production of light-duty EVs expected to increase from 0.3 to 1.5 million units from 2020 to 2030 (APC, 2021) and the UK a significant exporter of automotives (Faraday, 2021), supply constraints run the risk of negatively impacting industry output.
REE are mined as a by-product of primarily base-metal mining (BGS, 2021), leading to a disconnect between demand and supply. Coupled with long lead-times for new supply (Petravratzi & Gunn, 2022), this has led to an increase in prices.
While the value of REE imports to the UK stood at just £28.5M in 2017 (including as concentrates, compounds), these materials enter into a raft of goods produced and consumed. Diverse applications include high-value applications in catalysts, electrical equipment and magnets (BGS, 2021) as well as various product processes (Marscheider-Weidemann et al. 2021).
Disassembly
A growing stock of EVs in the UK presents a potential supply opportunity in the face of high levels of demand and price increases. In the UK's Critical Minerals Strategy (BEIS, 2022), accelerating to a more circular economy with improved end-of-life treatment has been put forward as a key strategy by which to lessen security of supply risks, improve environmental performance and meet societal objectives (Bloodworth et al. 2019).
Barriers to this include relatively low volumes of REEs in products, frequently immature technologies (such as for sorting and recycling) and supply chains to recover materials linked to high costs and exacerbated by gaps in product and component data. Some data systems do exist such as the International Dismantling Information System.
While magnets have the potential to outlive its assembly through reuse, remanufacture and recycling, the cost of their dismantling and removal remains high and limits recycling. While data on the recovery of REEs is scant, they are generally understood to be managed within predominantly linear value chains. Lusty et al. (2021) assume an EOL-RIR of 5.5% for REEs based on an average of light REE and heavy REE recycling rates published by the European Commission (2020).
Legal and regulatory
As a result of policy shift to electric mobility, the number of electric vehicles in use is continuously leading to greater volume of EV lithium-ion batteries coupled with issues around capacity, transport, safety and waste shipment regulations. In order to address this challenge a set of policy and regulatory interventions are required:
- Developing clear regulation and policy on re-use and re-purposing, including the influencing of contractual and ownership models (e.g. battery leasing schemes) for EV batteries to facilitate recycling and second-use. This intervention will assist in developing appropriate business models that will promote re-use and safe and effective life-management of EV batteries. An important precondition is developing clear legal definitions of what “re-use”, “repurposing” and “recycling” entail which should be done withing wider endeavour to revisit the definition of waste under the Waste Framework Directive.
- Developing extended producer responsibility (EPR) regulations that support a move to a circular economy model, ensuring safe and effective re-use of EV batteries, with increasingly robust recycling targets. This regulatory intervention will assist in increasing the recycling and recovery of material withing EV batteries.
- Developing eco-design criteria for recycling and remanufacturing, including restrictions on the use of hazardous substances and promotion of designs that allow easy separation of parts. This regulatory intervention will facilitate dismantling and component separation leading to greater economic viability of recycling.
- Introducing mandatory chemistry labelling requirements for lithium-ion batteries to enable end-of-life lithium batteries to be easily and safely sorted and separated for recycling in specific groups. Currently there is no legal requirement to label the cathode chemistry on a battery pack which is coupled with a variety of different technologies on the market. This measure will facilitate separation of batteries at the end of their life and recycling of batteries containing same or similar properties.
Critical materials and components
Neodymium (NdFeB) permanent magnets, two-thirds of which input to automotive, consumer electronic, wind turbine and air-conditioning applications (Roskill, 2019), are associated with relatively high performance characteristics which make them difficult to substitute given many low carbon technologies currently stretch the boundaries of technical performance in the short-term (BEIS, 2022). The magnets, an output of a relatively complex value chain on the inbound side but whose rate of recovery at end-of-life remains woefully low.
“Currently, there is little to no visibility in existing public data sources of these critical materials or component streams at the post-use phase as they are combined and aggregated into more complex products and assemblies…Accordingly, there is no consistent or standardised way to track or monitor stocks and flows of REE materials or permanent magnets.” Combining public data at the product-level on inflows and stocks with data on bill of materials to estimate content. A high level of variability between vehicle models and years for REE content coupled with small amounts of primary data made generalising REE content in vehicles, including those electric, more difficult (Løvik et al. 2021). CE-Hub working through data trust frameworks to improve insights into quantity, quality and location of these materials in addition to value-retention and resource productivity benefits.
Bill of materials of electric vehicles (EVs)
The transition to electric vehicles (EVs) would require access to significant quantities of technology metals, essential for the manufacture of EV components, such as electric powertrains and batteries. It is vital to understand different types of electric vehicles, electric traction motors and their composition of technology metals because the changing composition of electric vehicles over time creates challenges for end-of-life management and monitoring the flows and stocks.
Types of electric vehicles
Hybrid electric vehicles (HEVs) and Plug-in hybrid electric vehicles (PHEVs) are powered by both an electric traction motor and a combustion engine. HEVs can only be charged through regenerative braking. PHEVs can be charged not only through regenerative braking, but also through external power sources (a home charge point or a public charging station). Battery electric vehicles (BEVs) are zero-emissions vehicles powered by rechargeable batteries and electric traction motors without petrol or diesel engine. Instead of being powered by electricity stored in a battery, another new type of EV is the hydrogen Fuel Cell electric vehicle (FCEV) which relies on the electrochemical reaction between hydrogen and oxygen in a fuel cell stack to produce electricity.
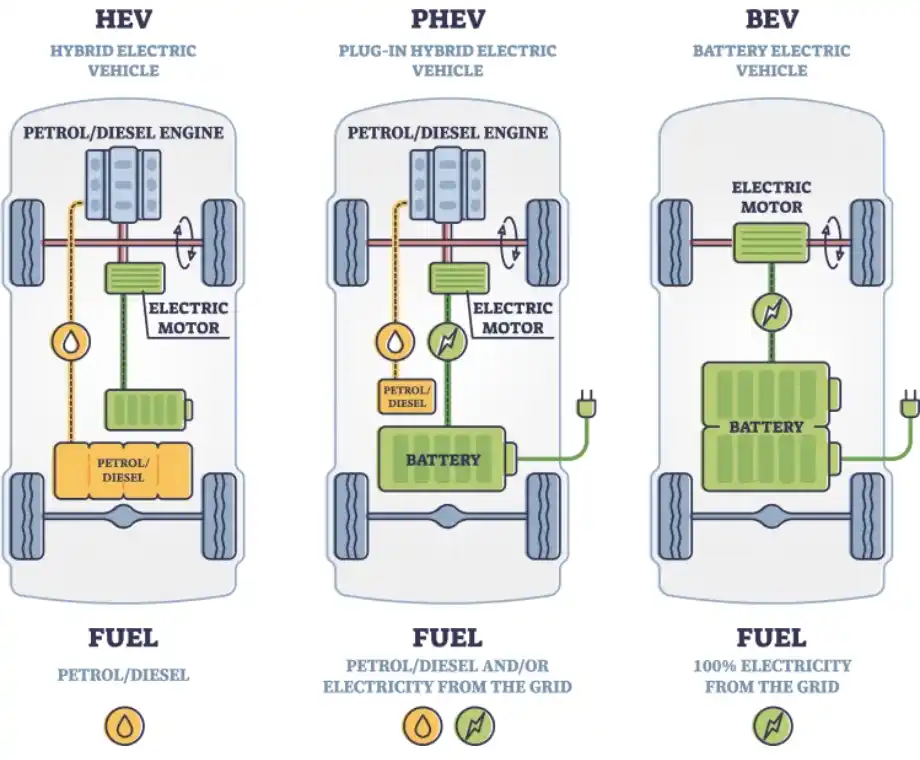
Electric traction motors
Electric traction motors used in EVs can be classified into direct current motors (DC) and alternating current motors (AC) (Figure 3). Permanent magnet motors, induction motors, and wound rotor motors are the main electric traction motors that dominate the EV market. Different model series of electric vehicles use different types of motors due to the cost of materials, power density, torque density and efficiency. Permanent magnet motors containing rare earth elements are the most commonly used motor, and their market share has been increasing in the past few years. In 2022, permanent magnet electric traction motors rose to 86% of the global EV market share over 84% in 2021 (IDTechEx, 2022).
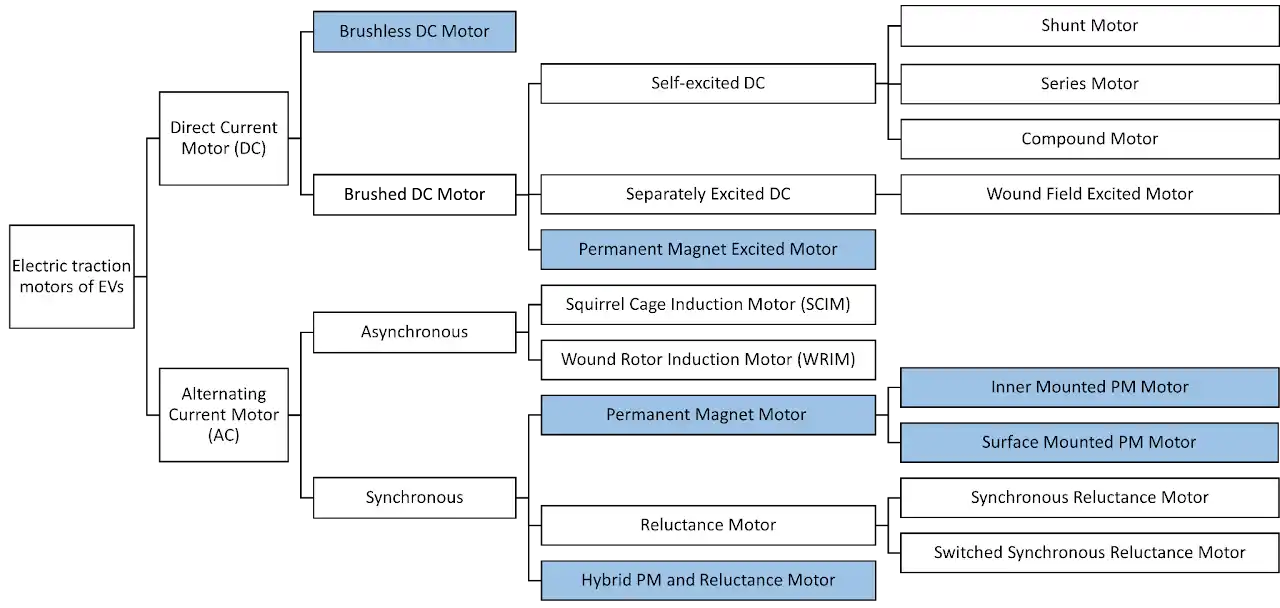
Translation into material stocks
Table 1 shows the amount of key technology metals in terms of mass in grams by different types of electric vehicles. The composition of different types of EVs varies due to the different drivetrains and batteries used in the EVs.
Metal | HEV and PHEV (Li-ion) | HEV and PHEV (NiMH) | BEV |
---|---|---|---|
Cobalt | 2,712 | 8,313 | 9,330 |
Dysprosium | 165.72 | 165.72 | 224.63 |
Lithium | 2,242 | 1.36 | 7,709 |
Manganese | 5,968 | 5,968 | 5,530 |
Neodymium | 552.79 | 2,631 | 749.3 |
Nickel | 16,049 | 82,832 | 55,724 |
Praseodymium | 51.48 | 2,129 | 98 |
Terbium | 13.62 | 13.62 | 29.93 |
Further reading
Ortego, A., Valero, A., Valero, A., & Restrepo, E. (2018). Vehicles and critical raw materials: A sustainability assessment using thermodynamic rarity. Journal of Industrial Ecology, 22(5), 1005-10151
https://doi.org/10.1111/jiec.12737
Iglesias-Émbil, M., Valero, A., Ortego, A., Villacampa, M., Vilaró, J., & Villalba, G. (2020). Raw material use in a battery electric car-a thermodynamic rarity assessment. Resources, Conservation and Recycling, 158, 104820.
Contact
For questions regarding the project please contact Dr Evi Petavratzi.